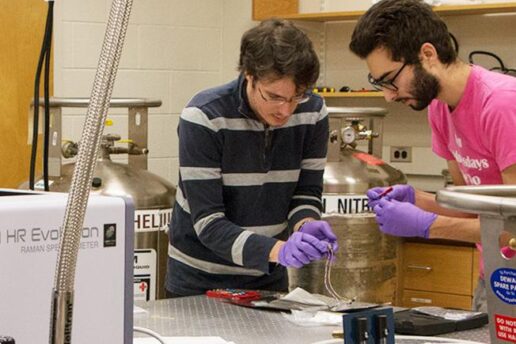
Better superconductors from ceramic copper oxides
Riccardo Comin seeks to elucidate the microscopic physics of high-temperature superconducting devices to advance their technological applications.
Medical magnetic resonance imaging, high-power microwave generators, superconducting magnetic energy storage units, and the solenoids in nuclear fusion reactors are very different technologies which all critically rely on the ability of superconducting materials to carry and store large electric currents in a compact space without overheating or dissipating large amounts of energy.
Despite their extraordinary properties, most superconducting materials present their own set of demands, such as the need to cool down to the temperature of liquid helium for medical MRIs. Still, superconductors are so efficient compared to everyday materials like copper that the cost of cooling them down with special cryogenic circuits is negligible compared to the energy saved from being converted — and ultimately wasted — in the form of heat, says Riccardo Comin, an assistant professor of physics.
“When you are trying to run a large current through a conventional circuit like one that’s made of copper, there will be a lot of dissipation into heat because of the finite electrical resistance of the material,” he says. “And that’s energy that just goes lost. Because superconductors can support flow of electrons without dissipation that means that you can run very large currents, known as supercurrents, through a superconductor, without the superconductor heating up to high temperatures.”
“You can inject a current in a superconductor and then just let it flow,” Comin says. “Then, a superconductor can act basically like a battery, but instead of storing energy as a voltage difference, which is what you have in a lithium ion battery, you store energy in the form of a supercurrent. Then you can extract and use that current, and it’s the same as pulling charge from a battery.”
What sets a superconductor apart from a conventional conductor is that, in the latter, you have to apply a potential between two different points to run a current through, but in the former, you can just set in motion the current and then remove the voltage, leave the system as is, and there will be a persistent current flowing through the material.
Comin explains further: “You have initiated a motion, or flow, of electrons, that will persist forever, protected from dissipation by the laws of quantum mechanics. It’s superfluid in the sense that the flow of electrons does not encounter resistance, or friction. Even if you remove the initial source that created that flow, it will continue unabated as in a frictionless electronic fluid.”
This electronic super fluidity is a quantum state of matter, so it behaves in a very exotic way that is different from classical physics, Comin says. It is already being used in many high-power applications requiring large currents or large magnetic fields.
Because superconductors can sustain very large currents, they can store a lot of energy in a relatively small volume. But even superconducting materials cannot sustain limitless electrical currents, and they can lose their special properties above a critical current density, which is in excess of 10 mega-amperes per square centimeter for state-of-the-art superconducting cables. By comparison, copper can carry a maximum current density of 500 amperes per square centimeter, which is same as the current density passed through a 100-watt tungsten wire light bulb.
While these critical currents where superconductivity turns off are known, what happens at the nanoscale inside the material as its approaches that critical condition is still unknown, yet it might hold the key to engineer better superconducting cables and devices, with even higher resilience.
Comin was one of three MIT researchers to win a U.S. Air Force Young Investigator Research Program grant this fall. The three-year, $450,000 award will allow Comin to pursue research into what happens to one particular superconducting material, yttrium barium copper oxide (YBCO) when it is driven at large currents.
“Studying the electrical response of a superconductor as one drives a large current through it is essential for characterizing superconducting circuits, but there is a lot of microscopic information of what’s happening inside the material that’s left to reveal,” he says. “The nanoscale physics of superconductors under operational conditions, namely when large currents are passed through them, is exactly what we’re interested in elucidating.”
“This is in a way a new direction where we’re not just studying the material in its undisturbed state, let’s say, just as a function of temperature, but without applying any sort of perturbation like a current or a field. Now we’re moving into a direction where we’re studying what happens in materials as they are driven at conditions of large currents, which are very close to those one would find inside a device or machine based on these superconducting circuits,” Comin explains.
Unlike niobium-tin alloys that require liquid helium cooling (about 4 kelvins) in MRI machines, YBCO superconducts at the somewhat higher temperature of liquid nitrogen. This is significant because liquid nitrogen (about 77 kelvins, or -320.4 degrees Fahrenheit) is both more abundant and considerably cheaper to use than helium, Comin says.
But there is another price to pay. Compared to a conventional metal or conductor like copper, which is ductile and easily shaped, YBCO is a brittle ceramic that has to be cast in two-dimensional layers on a base similar to old-fashioned cassette recording tapes.
“It has a layered structure, so it forms two-dimensional atomic sheets that are weakly coupled between them, and it’s very different from what a conventional metal would look like,” Comin says. Comin will study the material in his lab at MIT as well as at National Laboratories while high current is applied to it around or even below liquid nitrogen temperatures.
Although superconductivity takes over at liquid nitrogen temperature, as the material is subjected to larger and larger electric fields, other electronic states, or phases, such as a charge density wave, begin to compete with superconductivity before it ceases.
“When you start to weaken superconductivity, other electronic phases start to wake up and they compete to take control over the material,” he says. He plans to explore how the balance shifts between the superconducting phase and these other parasitic phases, as superconductivity weakens at high currents.
“Do these (other phases) start to take over or do they remain dormant?” Comin asks. “In one case, electrons want to flow without dissipation, and in the other case, they are stuck in place and cannot move around, like a car in a traffic jam.”
Instead of being able to freely move like they do in a superconductor, without any dissipation, electrons in a charge density wave tend to sit in some regions and stay there.
“There are some regions that have more electrons, some other regions that have fewer electrons, so if you try to visualize the spatial organization of these electrons, you see that it sort of wiggles as a wave,” Comin explains. “You can imagine a landscape of sand ripples on a dune. What drives the electrons to organize into a superfluid state rather than forming these static, wave-like patterns is not really known and it’s what we hope to discover under those critical conditions where the superconductor starts to yield to these other competing tendencies.”
The ultimate goal of this research effort is to elucidate how a persistent current, or supercurrent, flows around non-superconducting regions hosting competing phases, when the latter start to proliferate near critical conditions.
“In this project, supported by the Air Force Office for Scientific Research, we hope to gain new insights on the nanoscale physics of these superconducting devices, insights that could be transferred onto future superconductor technologies,” Comin says.