Strong Interactions and Nuclear Theory
The challenge of understanding strong interactions is a unifying theme that cuts across many areas of CTP research and also plays a central role in aspects of the physics of condensed matter, nuclear astrophysics and ultracold atoms, in particular in ultracold gases of fermionic atoms whose coupling has been tuned to be as strong as possible. The strong interactions among quarks and gluons, described by Quantum Chromodynamics (QCD), are particularly important because they exhibit many characteristic and challenging features of a strongly coupled theory while at the same time they are described at short length scales by a well-understood and well-tested theory, QCD, which is a central part of the Standard Model.
Understanding strong QCD interactions is crucial to interpreting collider searches for new short distance physics, within and beyond the Standard Model, as well as to understanding the properties of the hot matter that filled the microseconds old universe and the dense matter in the centers of neutron stars. These interactions are also the key to understanding how quarks and gluons form protons, neutrons, and other hadrons – which were the earliest complex structures formed in the universe – and subsequently nuclei. QCD provides a defining example of a theory in which the entities and phases that it describes do not resemble the elementary constituents of which they are made. This feature is characteristic of strongly interacting systems in many areas of physics and makes them both interesting and challenging.
Effective Field Theory
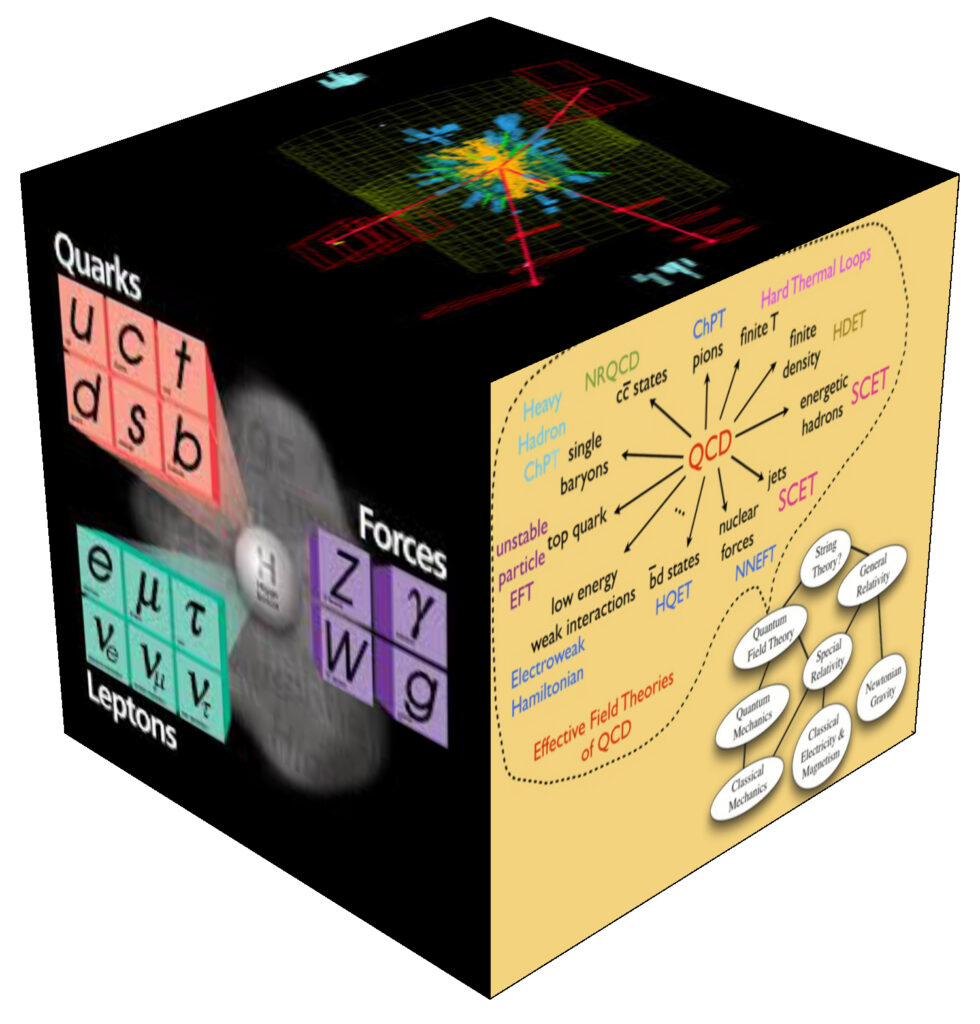
Effective field theory provides a crucial tool both for understanding QCD and for probing the short distance description of nature embodied by the electroweak part of the Standard Model. In recent years, the number of physical phenomena successfully described by effective field theory methods has been rapidly expanding, and MIT faculty have made crucial contributions to these developments. This includes Iain Stewart’s development of the soft-collinear effective theory, which enables rigorous field theory based calculations of cross-sections and decay rates, particularly those involving energetic jets formed by high energy quark and gluon collisions. This formalism has enabled improvements in precision by a factor of ten for cross-section calculations, and has made calculations for a broader range of sophisticated measurements theoretically tractable. Recent applications include developing observables for the future Electron-Ion Collider that can provide much more precise dynamic probes of the transition from quarks and gluons into hadrons, namely confinement and hadronization, and can probe key aspects of the internal workings of the proton and other hadrons. Other examples of Iain Stewart’s work include probing fundamental symmetries like the Standard Model description of CP violation and weak decays, and precisely determining essential parameters like the strong coupling constant. He also uses effective field theory tools to improve our understanding of two frontiers in gauge field theory: its infrared behavior at subleading power and the structure of cross-sections and amplitudes in the forward limit.
Effective field theory also has important applications for lattice QCD calculations, where it can be used to disentangle short and long distance QCD effects and account for a number of systematic effects. A recent example is Iain Stewart and Phiala Shanahan’s development of calculations of transverse momentum dependent parton distribution functions. Other recent developments using an effective theory formalism include Tracy Slatyer and Iain Stewart’s calculations of annihilation cross-sections needed in the search for heavy dark matter by indirect detection and Hong Liu’s analysis of dissipative fluids.
In recent years there has also been a renaissance in the realm of jet physics, including both our understanding of jet properties and the invention of new jet observables, where both Jesse Thaler and Iain Stewart have played important roles, for example providing theoretical tools and results that are now used in new physics searches and Higgs analyses at the LHC, including those carried out by Philip Harris and Christoph Paus as part of the CMS collaboration. Thaler has also been a key pioneer involved in developing methods that for the first time make it possible to use the substructure of a jet to determine its parentage, for example whether it came from a gluon, a quark, a boosted W boson, or even a supersymmetric squark.
Lattice Gauge Theory
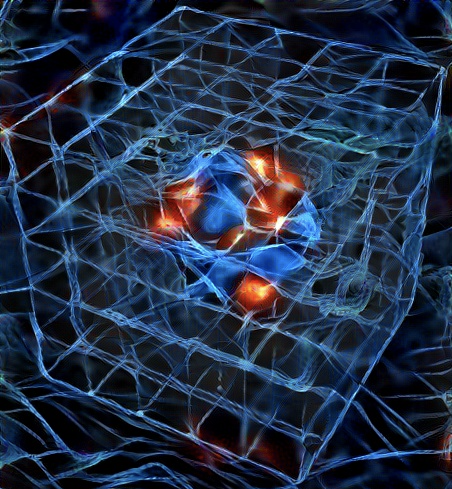
The low-energy physics of hadrons and nuclei arises from the same Standard Model that describes the quark interactions that are probed at high-energy colliders, but it requires different theoretical methods. William Detmold, John Negele, and Phiala Shanahan study QCD at low energies from first principles using a lattice field theory approach and thereby understand how QCD, whose fundamental degrees of freedom are quarks and gluons, gives rise to the rich and complex structure of protons, neutrons, and eventually nuclei. By employing innovative analytic and computational methods, including machine learning, they solve complex problems in QCD that are not amenable to other techniques.
Will Detmold’s research centers on obtaining quantitative understanding of how the complexity of nuclei emerges from their underlying quark and gluon degrees of freedom, and of the dynamics of the rearrangement of the light quarks and gluons that occurs when a heavy quark decays, for example at particle colliders such as the LHC where LNS colleagues Eluned Smith and Mike Williams and measure these decays using the LHCb detector. Will Detmold and Phiala Shanahan’s advances in the QCD study of nuclei have the potential to transform nuclear physics as, coupled to effective field theories of nucleon interactions, where Iain Stewart has made important contributions, they provide a path towards ab initio calculations of nuclear processes with fully quantifiable uncertainties. Recent highlights include the calculation of the weak decay rates of light nuclei, the modification of quark structure within a nucleus, and first efforts to address nuclear transitions needed to interpret neutrinoless double-beta decay experiments from first principles.
Phiala Shanahan’s work has led to a new understanding of the role of gluons in nucleons and nuclei and she and John Negele have led important investigations of the three-dimensional structure of the nucleon. These studies are providing impetus, predictions and benchmarks for new experiments at Jefferson Lab and at the future Electron-Ion Collider. She is also pioneering the application of principled machine learning to lattice field theory, developing novel algorithms to enable calculations that are intractable by conventional means. Her exciting new machine learning methods show promise to replace an algorithm that has been the foundation of lattice QCD for three decades. At the same time, the methods that she is developing are finding applications in diverse areas such as in studies of protein folding. Will Detmold and Phiala Shanahan are also engaged in exploratory investigations of the ways in which quantum computing and other novel architectures can be harnessed to solve problems in lattice QCD. Detmold, Negele and Shanahan also perform carefully quantified calculations of unmeasured properties of nucleons and nuclei that are needed in experimental searches for dark matter and other new physics. They are key members of a national initiative exploiting the country’s most powerful computers for lattice QCD, and also use large-scale resources at MIT.
Hot QCD
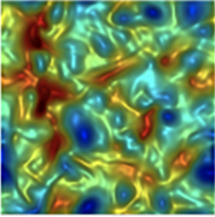
At high enough temperature and/or density, QCD describes various phases of matter in which the quarks and gluons do not coalesce into hadrons or nuclei. Understanding these liquids requires linking particle and nuclear physics, cosmology, astrophysics and condensed matter physics. Experimentalists including Gian Michele Innocenti, Yen-Jie Lee, Gunther Roland and Bolek Wyslouch have used heavy ion collision experiments at the Relativistic Heavy Ion Collider (RHIC) and the LHC to show that the hot quark-gluon plasma that filled the microseconds-old universe is a strongly coupled liquid. Understanding the properties of this new phase of matter and how it emerges from QCD is a central challenge for the coming half-decade, motivating a luminosity upgrade at the LHC and the construction of the new sPHENIX detector at RHIC which began taking data in Spring 2023.
Krishna Rajagopal is incorporating insights obtained via gauge/string duality, perturbative QCD methods, and hydrodynamics in modeling how jets produced in heavy ion collisions are modified via their passage through liquid quark-gluon plasma and how the wakes they leave behind in the droplet of liquid relax and evolve, discerning the most effective ways to use measurements of how the substructure of jets gets modified as individual quarks and gluons within them scatter off individual quarks and gluons from the QGP to gain an understanding the microscopic structure of this strongly coupled liquid as well as how it forms and hydrodynamizes as remarkably quickly as it does. The longer term challenge to theorists is to use the data to gain an understanding of how a strongly coupled liquid, which shows no signs of the individual particles of which it is made, can emerge from QCD. This quest resonates with challenges that are central to contemporary condensed matter physics, where Hong Liu has used gauge/string duality techniques developed to study quark-gluon plasma to gain insights into superfluids, and some of the most interesting and most puzzling materials, including “strange metals”. It also connects with Jesse Thaler‘s work on understanding the substructure of jets from first principles using machine learning techniques and on defining observables that characterize jet substructure — as well as its modification via passage through a droplet of QGP.
Krishna Rajagopal has also analyzed the critical point in the QCD phase diagram and the interplay of hydrodynamics and fluctuations near it. He has proposed signatures for its experimental detection, showing how to use data coming soon from the recently completed collision-energy scan at RHIC to search for the critical point in a large region of the QCD phase diagram. Rajagopal and Frank Wilczek have previously analyzed the properties of the superfluid, color superconducting, quark matter that may lie at the centers of neutron stars, providing a clear understanding of the properties of such matter at very high densities.