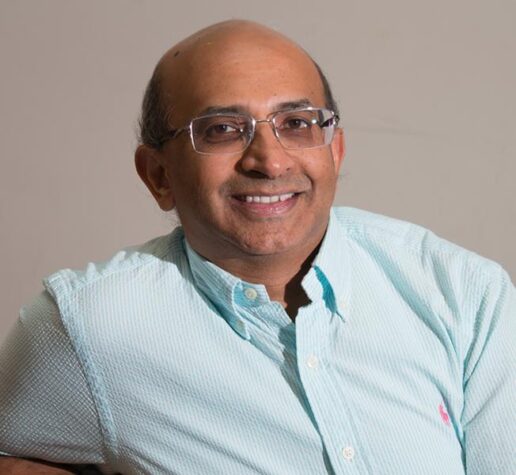
Faculty highlight: Senthil Todadri
A quest to understand superconductivity leads MIT theoretical physicist Senthil Todadri to discoveries about new magnetic materials called quantum spin liquids.
Mother nature is like a restless child who fidgets even when at rest, because electrons are never completely at rest, even at the coldest temperatures, says Professor Senthil Todadri, a theoretician in the MIT Department of Physics. Imagine pushing a pendulum hanging from a clock. It will swing back and forth, but eventually it will come to a complete stop so that it has a velocity of zero, but we also can see that it has a definite position in space. In the quantum world of electrons, knowing both of these properties, velocity and position, with ultimate precision is forbidden by the Heisenberg uncertainty principle.
“Quantum mechanically, there is still some motion even in the default state, what we call the ground state,” Senthil says. (Although his legal name is Senthil Todadri, he publishes under the name T. Senthil.) “It’s unavoidable motion that’s there, even in the default state, the lowest energy state that a quantum system can find itself in. There is still some motion.” And this basic fact of nature underlies a variety of unusual behaviors of electrons in materials. Explaining these hard-to-observe physical conditions such as new forms of magnetism is Senthil’s life’s work.
Unraveling magnetism
Everyday permanent magnetism in materials such as iron, where opposite poles attract and like poles repel, has been known for some 2,600 years, Senthil notes; these are called ferromagnets, derived from the Latin word for iron. “A ferromagnet is something in which the electrons inside the material, they have tiny magnetic moments, and those tiny magnetic moments all line up together to form a giant magnet, and that’s what we see in the ordinary world as a ferromagnet,” he says.
Less than a century ago, physicists identified a new kind of emergent magnetism in materials that are called antiferromagnets. In an antiferromagnet, tiny magnetic moments of individual electrons are frozen in space, but the pattern, or direction, of these tiny moments, oscillates in space on an atomic length scale. “From a macroscopic point of view, if you take an antiferromagnet, it’s very hard to know it has any kind of magnetism at all,” he says. That’s because this magnetism varies on a scale of one angstrom to a few angstroms, which is the size of just a few atoms, and technology to detect it didn’t become available until the 20th century.
“But now we know that antiferromagnetism is by far the most common form of magnetism. If you look around in magnetic materials, there are many, many more antiferromagnets than there are ferromagnets. It’s just that they are much harder to detect,” Senthil explains.
While it can be studied by itself, magnetism is intimately related to electricity, since every electron, including a magnetic one, carries an electric charge. A different class of materials, called superconductors, lose their resistance to electricity at very cold temperatures and there textbook analysis breaks down, says Senthil. As they are being chilled to a temperature of about 100 kelvins (-279.67 degrees Fahrenheit), these materials first show unusual metallic behavior, then become superconducting. Among materials that have been discovered to superconduct, many don’t do so until they reach a range from about 4 kelvins down to a fraction of 1 kelvin, or nearly absolute zero, the coldest possible temperature. So in the world of physics, superconducting at 100 kelvins is spoken of as high-temperature.
“From a fundamental science point of view what makes them remarkable is they seem to violate almost all of our textbook understanding of how electrons behave inside a solid,” Senthil explains. “Before they become superconducting, when the material is so hot that it’s not a superconductor yet, it’s a metal, but it’s a really unusual metal, and it’s out of this unusual metal that the superconductor is born.”
“Many of us think that understanding this unusual metal will give us a clue as to why the system is superconducting,” he says. “One of the hopes is that once we understand that clue, we can then have sensible ideas on what kinds of other materials might superconduct at relatively high temperatures and eventually maybe the field will discover room temperature superconductivity.”
These magnetic and superconducting behaviors appear to be intimately connected. One such material, lanthanum copper oxide, becomes an antiferromagnet at about 27 degrees Celsius (80 F). “Certainly above room temperature in Boston right now,” Senthil says on a chilly winter day. “This is a very famous material. It’s famous because if you take this material and you remove electrons from this material, using chemical methods, it’s precisely this material that becomes one of those superconductors that I mentioned before. If you remove electrons, it losses its magnetism, but it becomes something else, it becomes a superconductor. Of course, it’s superconducting only at temperatures much colder than room temperature.”
“The thing I would really like to understand — that’s been a goal for more than 10 years now — is to understand these metals that defy the textbook description of a metal. In some sense, to me that’s the most outstanding challenge in this entire field, and over the years, we’ve been making slow but steady progress, not just I, myself, but the community as a whole, but I would really like that progress to accelerate. That’s my home base; that’s the problem I am always thinking about. Everything else almost feels like I am doing on the side, but that’s hard. There has been progress, enough to keep me encouraged, but not enough for me to jump up and down. So that’s my pet project. That’s what I see as the main thing I feel like I want to have done by the time I’m ready to retire,” Senthil reveals.
Random tumbling
Yet another form of magnetism in solid materials is the quantum spin liquid, a name for magnetism drawn by analogy from fluid materials. In a spin liquid, Senthil says, individual electrons still have magnetic moments but they are randomly tumbling around all the time. “If you took a snapshot of what the magnetic moments are doing, different electrons with spins will be pointing in different directions, and if you took a different snapshot, the pattern would be completely different. So as a function of time, it’s moving all over the place, so there is no net magnetic moment. It’s neither a ferromagnet nor an antiferromagnet. We describe that by saying there is no magnetic ordering. … That’s what’s called a spin liquid,” Senthil says.
Although there is no magnetic ordering, electrons in these spin liquids possess a property that no other form of magnetism has: Their magnetic moments are entangled quantum mechanically with the magnetic moments of other electrons far away from them. Quantum entanglement is one of the most counterintuitive concepts in physics. Its essence is that quantum mechanical systems that are separated from each other spatially still have some sort of contact with each other, what Einstein called “spooky action at a distance.” “The presence of quantum entanglement between distant parts of my sample, that is unusual, and it’s unprecedented in magnetism. So this is a new chapter in the study of magnetism. It leads to all kinds of unusual, bizarre phenomena that can potentially happen inside a solid,” Senthil explains.
An example of something unusual that can happen in these materials is that the electron may split into fractional pieces. How can that be when an electron is supposed to one of the fundamental particles of the universe? “An electron is supposed to be a fundamental particle in the vacuum of the universe, but inside a solid, the presence of long-distance quantum entanglement, enables the system to behave as some soup in which there are particle-like objects that move, but these objects are fractions of the electron,” he says. “What makes all these phenomena possible inside a system that looks very simple? It’s a collection of magnetic moments. What makes it possible is the long distance quantum mechanical entanglement that’s present between the localized magnetic moments. So I’ve been studying these kinds of magnetic matter. Not only is it fascinating by itself as a phenomenon, it turns out that studying these kinds of matter leads to all kinds of insights into other problems in condensed matter physics.”
In a February 2016 Physical Review B paper with Chong Wang PhD ’15, who now is a postdoc at the Society of Fellows at Harvard University, Senthil explored three-dimensional quantum spin liquids. Thinking about these unusual states of magnetism helped them map the connections between topological insulators, superconductors, and quantum spin liquids. “There are some deep connections between many different phenomena in the field in many different kinds of systems that we realized only recently just in 2015, 2016, which ended up in some cases solving, in some cases showing the way forward, on questions that have been open for more than 20 years in the field. So there is enormous progress that has come about in theoretical physics as a whole because of thinking about these kinds of novel states of magnetism,” he says.
Contradictory behavior
In particular, Senthil and Wang clarified how a particular metallic system chilled to very low temperatures in the presence of a large magnetic field can display the seemingly contradictory behavior of having both an overall current running at a right angles to an applied voltage while at the same time carrying charged particles that were shown in experiment to move in a straight line with the voltage. A long-standing theory from the early ’90s by co-authors Patrick A. Lee at MIT, Bertrand I. Halperin at Harvard and Nicholas Read at Yale University proposed that this phenomenon could be explained by thinking of each charge carrier as having an electron that attaches to itself a bit of magnetic flux, so that even though each such composite particle moves in a straight line, it carries both an electrical charge and a magnetic flux. This movement of magnetic flux produces an electric field in the perpendicular [or transverse] direction. But the Lee-Halperin-Read theory couldn’t explain a kind of physical symmetry in this system known as particle-hole symmetry, Senthil says. A hole, perhaps most familiar in the context of semiconducting materials, is the absence of an electron in an atom where one is expected. Symmetry means that whether you view the system as a collection of electrons or view it as a collection of holes, the system is the same. “These are just two different viewpoints on what’s really the same system,” Senthil says.
In experiments, replacing electrons with holes in this system didn’t make a physical difference, so it is considered to be in electron-hole symmetry. Physicists model electrons mathematically through a complicated formula known as a wave function, which incorporates the electrons’ properties such as spin and momentum. Senthil says he and Wang were motivated by an idea proposed by Dam T. Son at the University of Chicago that this could be explained by thinking of the composite particle as carrying a “spin” that is locked into some definite angle to its momentum. A peculiar occurrence in quantum physics is that when spin of this composite particle, just like the electron spin itself, is rotated in a full circle, the particle’s quantum mechanical wave function turns from a positive number to a negative number. By coupling spin to momentum in this system, Senthil explains, “What we now understand in this story is that these objects that move in straight lines inside this medium, inside this two-dimensional collection of electrons in a strong field, that they have this feature that if you rotate their momentum on a full circle, the wave function changes sign, so that’s a different theory from the older theory.”
The technical term for these spin-momentum coupled particles is Dirac particles, from the Dirac equation that quantifies their quantum state. “Going from electrons to holes, it turns out, flips the direction of the momentum of these Dirac particles, and it also has the effect of flipping, therefore, the direction of the spin, because the spin of this particle is tied to the momentum. So the momentum changes sign, the spin changes sign, so that’s all that happens,” he says. But importantly, it explains how the electron-hole symmetry acts in this system. Wang and Senthil derived this solution from their understanding of other phenomena in condensed matter physics, such as topological insulators and quantum spin liquids. “In the process, we ended up learning a lot about all of these different systems,” Senthil says.
Senthil notes that these ideas also were pursued independently by Max Metlitski, who recently joined the MIT Department of Physics faculty as assistant professor in the Condensed Matter Theory group, and Harvard professor of physics Ashvin Vishwanath. “These are friends of mine who it turns out were working on the same thing at the same time independently,” Senthil says. Vishwanath previously was a Pappalardo Fellow at MIT, serving as a postdoctoral associate in physics with Senthil.
Research group
Senthil supervises a small group of graduate students: Michael Pretko and Liujun Zou, who are working on quantum spin liquids, and Yahui Zhang, who is working on unconventional metallic states of matter through numerical calculations. “In experimental groups, the PI [principal investigator] tells the group what to work on. In theory groups, there is a lot more freedom. If a student comes to me and says, look, I have this idea, I encourage them to work on it, even if I am not working on it myself. … It’s a great thing for students to come up with their own ideas,” Senthil says. Senthil also works with Pappalardo Fellows Inti Sodemann and Itamar Kimchi and Moore Fellow Sam Lederer and advises them on their work on magnetism, unconventional metals, and superconductivity.
This spring, Senthil will teach an advanced graduate course on Many Body Quantum Mechanics. Over the past four years, he taught quantum mechanics for first-year graduate students.
Senthil is married and has two daughters, ages 7 and 14. “Whenever I find time, which is getting harder and harder, I try to read,” he says.