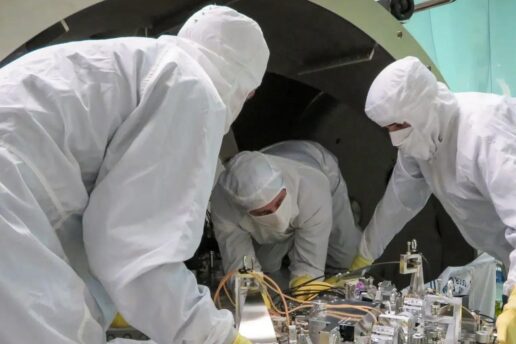
How scientists are using quantum squeezing to push the limits of their sensors
Fuzziness may rule the quantum realm, but it can be manipulated to our advantage.
When two black holes spiral inward and collide, they shake the very fabric of space, producing ripples in space-time that can travel for hundreds of millions of light-years. Since 2015, scientists have been observing these so-called gravitational waves to help them study fundamental questions about the cosmos, including the origin of heavy elements such as gold and the rate at which the universe is expanding.
But detecting gravitational waves isn’t easy. By the time they reach Earth and the twin detectors of the Laser Interferometer Gravitational-Wave Observatory (LIGO), in Louisiana and Washington state, the ripples have dissipated into near silence. LIGO’s detectors must sense motions on the scale of one ten-thousandth the width of a proton to stand a chance.
LIGO has confirmed 90 gravitational wave detections so far, but physicists want to detect more, which will require making the experiment even more sensitive. And that is a challenge.
“The struggle of these detectors is that every time you try to improve them, you actually can make things worse, because they are so sensitive,” says Lisa Barsotti, a physicist at the Massachusetts Institute of Technology.
Nevertheless, Barsotti and her colleagues recently pushed past this challenge, creating a device that will allow LIGO’s detectors to detect far more black hole mergers and neutron star collisions. The device belongs to a growing class of instruments that use quantum squeezing—a practical way for researchers dealing with systems that operate by the fuzzy rules of quantum mechanics to manipulate those phenomena to their advantage.
Physicists describe objects in the quantum realm in terms of probabilities—for example, an electron is not located here or there but has some likelihood of being in each place, locking into one only when its properties are measured. Quantum squeezing can manipulate the probabilities, and researchers are increasingly using it to exert more control over the act of measurement, dramatically improving the precision of quantum sensors like the LIGO experiment.
“In precision sensing applications where you want to detect super-small signals, quantum squeezing can be a pretty big win,” says Mark Kasevich, a physicist at Stanford University who applies quantum squeezing to make more precise magnetometers, gyroscopes, and clocks with potential applications for navigation. Creators of commercial and military technology have begun dabbling in the technique as well: the Canadian startup Xanadu uses it in its quantum computers, and last fall, DARPA announced Inspired, a program for developing quantum squeezing technology on a chip. Let’s take a look at two applications where quantum squeezing is already being used to push the limits of quantum systems.
Taking control of uncertainty
The key concept behind quantum squeezing is the phenomenon known as Heisenberg’s uncertainty principle. In a quantum-mechanical system, this principle puts a fundamental limit on how precisely you can measure an object’s properties. No matter how good your measurement devices are, they will suffer a fundamental level of imprecision that is part of nature itself. In practice, that means there’s a trade-off. If you want to track a particle’s speed precisely, for example, then you must sacrifice precision in knowing its location, and vice versa. “Physics imposes limits on experiments, and especially on precision measurement,” says John Robinson, a physicist at the quantum computing startup QuEra.
By “squeezing” uncertainty into properties they aren’t measuring, however, physicists can gain precision in the property they want to measure. Theorists proposed using squeezing in measurement as early as the 1980s. Since then, experimental physicists have been developing the ideas; over the last decade and a half, the results have matured from sprawling tabletop prototypes to practical devices. Now the big question is what applications will benefit. “We’re just understanding what the technology might be,” says Kasevich. “Then hopefully our imagination will grow to help us find what it’s really going to be good for.”
LIGO is blazing a trail to answer that question, by enhancing the detectors’ ability to measure extremely tiny distances. The observatory registers gravitational waves with L-shaped machines capable of sensing tiny motions along their four-kilometer-long arms. At each machine, researchers split a laser beam in two, sending a beam down each arm to reflect off a set of mirrors. In the absence of a gravitational wave, the crests and troughs of the constituent light waves should completely cancel each other out when the beams are recombined. But when a gravitational wave passes through, it will alternately stretch and compress the arms so that the split light waves are slightly out of phase.
The resulting signals are subtle, though—so subtle that they risk being drowned out by the quantum vacuum, the irremovable background noise of the universe, caused by particles flitting in and out of existence. The quantum vacuum introduces a background flicker of light that enters LIGO’s arms, and this light pushes the mirrors, shifting them on the same scale as the gravitational waves LIGO aims to detect.
Barsotti’s team can’t get rid of this background flicker, but quantum squeezing allows them to exert limited control over it. To do so, the team installed a 300-meter-long cavity in each of LIGO’s two L-shaped detectors. Using lasers, they can create an engineered quantum vacuum, in which they can manipulate conditions to increase their level of control over either how bright the flicker can be or how randomly it occurs in time. Detecting higher-frequency gravitational waves is harder when the rhythm of the flickering is more random, while lower-frequency gravitational waves get drowned out when the background light is brighter. In their engineered vacuum, noisy particles still show up in their measurements, but in ways that don’t do as much to disturb the detection of gravitational waves.“ You can [modify] the vacuum by manipulating it in a way that is useful to you,” she explains.
The innovation was decades in the making: through the 2010s, LIGO incorporated incrementally more sophisticated forms of quantum squeezing based on theoretical ideas developed in the 1980s. With these latest squeezing innovations, installed last year, the collaboration expects to detect gravitational waves up to 65% more frequently than before.
Quantum squeezing has also improved precision in timekeeping. Working at the University of Colorado Boulder with physicist Jun Ye, a pioneer in atomic clock technology, Robinson and his team made a clock that will lose or gain at most a second in 14 billion years. These super-precise clocks tick slightly differently in different gravitational fields, which could make them useful for sensing how Earth’s mass redistributes itself as a result of seismic or volcanic activity. They could also potentially be used to detect certain proposed forms of dark matter, the hypothesized substance that physicists think permeates the universe, pulling on objects with its gravity.
The clock Robinson’s team developed, a type called an optical atomic clock, uses 10,000 strontium atoms. Like all atoms, strontium emits light at specific signature frequencies as electrons around the atom’s nucleus jump between different energy levels. A fixed number of crests and troughs in one of these light waves corresponds to a second in their clock. “You’re saying the atom is perfect,” says Robinson. “The atom is my reference.” The “ticking” of this light is far steadier than the vibrating quartz crystal in a wristwatch, for example, which expands and contracts at different temperatures to tick at different rates.
In practice, the tick in the Robinson team’s clock comes not from the light the electrons emit but from how the whole system evolves over time. The researchers first put each strontium atom in a “superposition” of two states: one in which the atom’s electrons are all at their lowest energy levels and another in which one of the electrons is in an excited state. This means each atom has some probability of being in either state but is not definitively in either one—similar to how a coin flipping in the air has some probability of being either heads or tails, but is neither.
Then they measure how many atoms are in each state. The act of measurement puts the atoms definitively in one state or the other, equivalent to letting the flipping coin land on a surface. Before they measure the atoms, even if they intend to wind up with a 50-50 mixture, they cannot precisely dictate how many atoms will end up in each state. That’s because in addition to the system’s change over time, there is also inherent uncertainty in the state of the individual atoms. Robinson’s team uses quantum squeezing to more reliably determine their final states by reducing these intrinsic fluctuations. Specifically, they manipulate the uncertainties in the direction of each atom’s spin, a property of many quantum particles that has no classical counterpart. Squeezing improved the clock’s precision by a factor of 1.5.
To be sure, gravitational waves and ultra-precise clocks are niche academic applications. But there is interest in adapting the approach to other, potentially more mainstream uses, including quantum computers, navigation, and microscopy.
The increased use of quantum squeezing is part of a wider technological trend toward higher precision—one that encompasses cramming more transistors on chips, studying the universe’s most elusive particles, and clocking the fleeting time it takes for an electron to leave a molecule. Squeezing benefits only measurements so subtle that the randomness of quantum mechanics contributes significant noise. But it turns out that physicists have more control than they think. They may not be able to remove the randomness, but they can engineer where it shows up.