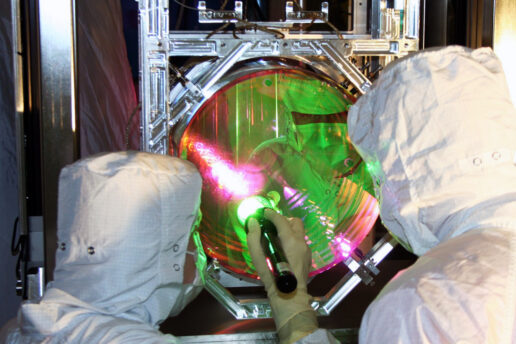
Physicists bring human-scale object to near standstill, reaching a quantum state
The results open possibilities for studying gravity’s effects on relatively large objects in quantum states.
To the human eye, most stationary objects appear to be just that — still, and completely at rest. Yet if we were handed a quantum lens, allowing us to see objects at the scale of individual atoms, what was an apple sitting idly on our desk would appear as a teeming collection of vibrating particles, very much in motion.
In the last few decades, physicists have found ways to super-cool objects so that their atoms are at a near standstill, or in their “motional ground state.” To date, physicists have wrestled small objects such as clouds of millions of atoms, or nanogram-scale objects, into such pure quantum states.
Now for the first time, scientists at MIT and elsewhere have cooled a large, human-scale object to close to its motional ground state. The object isn’t tangible in the sense of being situated at one location, but is the combined motion of four separate objects, each weighing about 40 kilograms. The “object” that the researchers cooled has an estimated mass of about 10 kilograms, and comprises about 1×1026, or nearly 1 octillion, atoms.
The researchers took advantage of the ability of the Laser Interfrometer Gravitational-wave Observatory (LIGO) to measure the motion of the masses with extreme precision and super-cool the collective motion of the masses to 77 nanokelvins, just shy of the object’s predicted ground state of 10 nanokelvins.
Their results, appearing today in Science, represent the largest object to be cooled to close to its motional ground state. The scientists say they now have a chance to observe the effect of gravity on a massive quantum object.
“Nobody has ever observed how gravity acts on massive quantum states,” says Vivishek Sudhir, assistant professor of mechanical engineering at MIT, who directed the project. “We’ve demonstrated how to prepare kilogram-scale objects in quantum states. This finally opens the door to an experimental study of how gravity might affect large quantum objects, something hitherto only dreamed of.”
The study’s authors are members of the LIGO Laboratory, and include lead author and graduate student Chris Whittle, postdoc Evan Hall, research scientist Sheila Dwyer, Dean of the School of Science and the Curtis and Kathleen Marble Professor of Astrophysics Nergis Mavalvala, and assistant professor of mechanical engineering Vivishek Sudhir.
Precision pushback
All objects embody some sort of motion as a result of the many interactions that atoms have, with each other and from external influences. All this random motion is reflected in an object’s temperature. When an object is cooled down close to zero temperature, it still has a residual quantum motion, a state called the “motional ground state.”
To stop an object in its tracks, one can exert upon it an equal and opposite force. (Think of stopping a baseball in mid-flight with the force of your glove.) If scientists can precisely measure the magnitude and direction of an atom’s movements, they can apply counteracting forces to bring down its temperature — a technique known as feedback cooling.
Physicists have applied feedback cooling through various means, including laser light, to bring individual atoms and ultralight objects to their quantum ground states, and have attempted to super-cool progressively larger objects, to study quantum effects in bigger, traditionally classical systems.
“The fact that something has temperature is a reflection of the idea that it interacts with stuff around it,” Sudhir says. “And it’s harder to isolate larger objects from all the things happening around them.”
To cool the atoms of a large object to near ground state, one would first have to measure their motion with extreme precision, to know the degree of pushback required to stop this motion. Few instruments in the world can reach such precision. LIGO, as it happens, can.
The gravitational-wave-detecting observatory comprises twin interferometers in separate U.S. locations. Each interferometer has two long tunnels connected in an L-shape, and stretching 4 kilometers in either direction. At either end of each tunnel is a 40-kilogram mirror suspended by thin fibers, that swings like a pendulum in response to any disturbance such as an incoming gravitational wave. A laser at the tunnels’ nexus is split and sent down each tunnel, then reflected back to its source. The timing of the return lasers tells scientists precisely how much each mirror moved, to an accuracy of 1/10,000 the width of a proton.
Sudhir and his colleagues wondered whether they could use LIGO’s motion-measuring precision to first measure the motion of large, human-scale objects, then apply a counteracting force, opposite to what they measure, to bring the objects to their ground state.
Acting back on back-action
The object they aimed to cool is not an individual mirror, but rather the combined motion of all four of LIGO’s mirrors.
“LIGO is designed to measure the joint motion of the four 40-kilogram mirrors,” Sudhir explains. “It turns out you can map the joint motion of these masses mathematically, and think of them as the motion of a single 10-kilogram object.”
When measuring the motion of atoms and other quantum effects, Sudhir says, the very act of measuring can randomly kick the mirror and put it in motion — a quantum effect called “measurement back-action.” As individual photons of a laser bounce off a mirror to gather information about its motion, the photon’s momentum pushes back on the mirror. Sudhir and his colleagues realized that if the mirrors are continuously measured, as they are in LIGO, the random recoil from past photons can be observed in the information carried by later photons.
Armed with a complete record of both quantum and classical disturbances on each mirror, the researchers applied an equal and opposite force with electromagnets attached to the back of each mirror. The effect pulled the collective motion to a near standstill, leaving the mirrors with so little energy that they moved no more than 10-20 meters, less than one-thousandth the size of a proton.
The team then equated the object’s remaining energy, or motion, with temperature, and found the object was sitting at 77 nanokelvins, very close to its motional ground state, which they predict to be 10 nanokelvins.
“This is comparable to the temperature atomic physicists cool their atoms to get to their ground state, and that’s with a small cloud of maybe a million atoms, weighing picograms,” Sudhir says. “So, it’s remarkable that you can cool something so much heavier, to the same temperature.”
“Preparing something in the ground state is often the first step to putting it into exciting or exotic quantum states,” Whittle says. “So this work is exciting because it might let us study some of these other states, on a mass scale that’s never been done before.”
This research was supported, in part, by the National Science Foundation.